A Report on Scientific Branch-Creation: How the Rockefeller Foundation helped bootstrap the field of molecular biology
This piece is noticeably longer (twice the length) than the others on this Substack. This is because 1) it is a practically-oriented historical follow-up on the ideas discussed in this Substack’s most popular piece on scientific branch-creation and 2) unlike the MIT series, there was not a reasonable way to break the research up into a series of distinct pieces. I took three times as long as usual to write and research this one, and I’m so excited to finally present it to you. Enjoy!
Since the explosion in physics knowledge of the early 1900s, driven largely by discoveries in quantum mechanics and relativity, many argue that only two scientific fields have been explosively productive. The first is computer science and electrical engineering — the history of which most readers of this Substack are familiar. The other is molecular biology.
The story of how (and why) molecular biology came to exist as a breakthrough field can, possibly more than anything, help us understand how we should be funding extremely young science. The story is largely centered around one organization, the Rockefeller Foundation, and to a significant extent, the vision of one man: Warren Weaver.
This piece details the story of how a second-rate physicist turned into (probably) the best scientific grant funder ever and is largely responsible for the creation of a field in spite of never producing a single paper in it or doing a tour of duty on one of its lab benches.
I will dive into the Rockefeller Foundation’s ridiculously anomalous results, how Warren Weaver’s thesis on scientific giving changed the foundation’s approach, evidence of this in Rockefeller’s annual budgets/reports, and first-hand accounts from what would later become the “molecular biologists” themselves speaking to just how important Weaver’s Natural Sciences Division was to the birth of the field.
This piece aims to help the reader under exactly how new branches can be created. There has been a previous Substack post by Samir Unni diving into how Rockefeller’s grant officers performed their most vital function, scouting young talent and projects. And I welcome you to check that out here if you’re interested. Overall, this piece focuses on what seems to me to be the primary factor that drove the Rockefeller Natural Sciences Division’s anomalous results: its commitment to scientific branch-creation at the expense of all other activities.
A quick note on “molecular biology” and “branch-creation”
I should quickly clarify a couple of terms: molecular biology and scientific branch-creation. Feel free to skip these sections if you’re already familiar. (Note: Scientific branch-creation was the subject of this blog’s most well-known piece, When do good ideas get easier to find?)
Molecular Biology
Crudely, molecular biology “seeks to understand the molecular basis of biological activity.” It can be described as the understanding of biological systems on an extremely small level — the kind of level that early 20th-century physicists and chemists were used to thinking on, but early 20th-century biologists were generally not. Watson and Crick’s discovery of the double-helical structure of DNA is considered an achievement in molecular biology, as is any scientific work having to do with their discovery that has followed. Modern tools like CRISPR and other heredity-based lab work can be broadly categorized as molecular biology.
Scientific Branch-Creation
In When do ideas get easier to find?, I outline Gerald Holton’s model of how scientific knowledge progresses — which was heavily dependent on branch-creation. This model was constructed based on what observed as a physicist throughout the early and mid-1900s.
A “branch” can be loosely understood as what happens when a new idea or tool — often combining several previously unrelated ideas or uncovering an extremely non-obvious insight — gets combined with existing tools/ideas to help researchers spawn a cluster of brand new ideas. An example of a very rich, extended branch that Holton used was spawned by I.I. Rabi’s work in developing the original molecular beam techniques — represented by the black box at the bottom of the following image which begins to branch upwards. This work by Rabi stimulated a group of wildly productive colleagues and students to explore these problems further, carving out a new sub-area of physics.
Holton strongly credited branch-creation, rather than branch continuation, with the majority of the gains in scientific knowledge. Holton uses a graph of early linear accelerators’ total energy gains to exemplify this point. The graph demonstrates that the exponential growth in total accelerator energy was driven by the explosive gains that came in the early years of new technologies (sub-branches), but the productivity of each technology slowed rapidly after its early years.
The slowing rate of progress for the individual curves did not matter much. While it was all too common for a single branch to rapidly curtail in research productivity, almost all new branches had explosive growth in their early stages. So, as long as new branches were being created frequently, scientific growth was explosive.
With that, let’s dive into it!
Rockefeller’s Anomalous Outcomes
Rockefeller’s results were, in a word, absurd.
In short:
In 1933, the Natural Sciences Division began to fund what it called “quantitative biology” at the expense of all other scientific areas. Weaver would not coin the term “molecular biology” until 1938. To put this in perspective, 1938 was 15 years before Watson and Crick discovered the structure of DNA which ushered in the “classical era” of molecular biology.
Even scientists in the mid-1940s, transitioning from fields like physics and biochemistry to molecular biology, still considered the move a gamble.
From 1954-1965, 18 Nobel Prizes were given out for molecular biology. 15 of them were beneficiaries of Rockefeller Foundation funding.
And, before you retort that “it’s easy to fund winners if they already have a track record,” the Foundation funded them, as Weaver noted, “on the average over nineteen years in advance” of winning the prize. In that era, people were often winning Nobel Prizes in their mid-to-late 40s…
Most of the influential scientists of the early field either directly credit the Rockefeller Foundation itself or one of the entities that were largely Rockefeller-funded with bootstrapping the early growth of the field — such as the Cold Spring Harbor Laboratory and its summer research program, the Caltech molecular biology research programs, or Max Delbrück himself. I’ll dive into the importance of all three later.
If you’re thinking, “anybody can get lucky once if there are enough organizations giving grants,”…Weaver would go on to do it again. In the early 1950s, just as the field of molecular biology was blooming with the discovery of DNA’s structure, Weaver began diverting much of his Division’s funding away from molecular biology and towards a young agricultural research program. This program would go on to be responsible for one of the greatest scientific discoveries of the 20th century in terms of human welfare gains: miracle rice.
If that’s a satisfactory rundown of the Rockefeller Natural Sciences Division’s accomplishments for you, you can skip ahead to the next section. The coming (long) quote from Weaver is fantastic, but not essential to understand the core of the piece.
For those who are interested, I felt I should also include Weaver’s own summary of why molecular biology is so important and why he thought his Division was largely responsible for its early growth, in his own words. He is a very humble man — as the rest of his autobiographical writing demonstrates — who did not have a tendency to aggrandize his accomplishments. However, when it came to some of his Natural Sciences Division’s successes, it was simply hard not to view his Division’s work as causal.
The following is an excerpt from Weaver’s autobiography, written in the late 1960s.
Although the new Rockefeller Foundation program in quantitative experimental biology was not started until I assumed, in February 1932, my duties as the Director of the Division of Natural Sciences, the record of grants indicated that we began rather promptly to find opportunities to finance promising research programs that were relevant to our program interests. In 1932, for example, we made the first of what turned out to be a long series of grants to the Biological Laboratory at Cold Spring Harbor, Long Island, New York. That institution began a series of summer symposia on quantitative biology, and these meetings played a critically important role in attracting to newer fields of biology a considerable number of brilliant young scientists, several of whom went on to furnish leadership in the new developments. This record is impressively set out in a volume published by the Cold Spring Harbor Laboratory.
Also in 1932 my division of the Rockefeller Foundation made the first of a considerable series of grants to the California Institute of Technology to help support the research program of Dr. Linus Pauling. The Foundation report for 1932, using language that might have seemed a little overoptimistic at the time, stated that Pauling’s “program in structural chemistry extends the technique of wave mechanics to the study of complex inorganic and organic molecules.”
But in 1951 Dr. Pauling magnificently substantiated that statement by publishing his famous theoretical deduction of the α-helix structure that occurs in proteins, a result which Sir W. Lawrence Bragg has called the “first example of a correct determination of atomic arrangement in biological substances.”
Soon after 1932 we began to support the researches of W.T. Astbury in England, a pioneer in the X-ray analysis of natural fibers, his early work being done on wool. And then we began making grants to a considerable number of physicists who were applying X-ray diffraction methods to the study of the structure of biologically important substances, particularly proteins.
I include a few details of the early grants made in the Rockefeller Foundation program for two reasons. First, I consider the emergence of the subject now regularly called molecular biology to be one of the greatest developments in the history of science. The triumphs to date of molecular biology have been largely in the field of genetics. But there is every reason to believe that molecular biology will now attack, and similarly conquer, other basic biological problems—those of immunology, of cellular growth and development (including cancer), and even some of the most basic aspects of the functioning of the central nervous system.
Second, I believe that the support which the Rockefeller Foundation poured into experimental biology over the quarter of a century following 1932 was vital in encouraging and accelerating and even in initiating the development of molecular biology. Indeed, I think that the most important thing I have ever been able to do was to reorient the Rockefeller Foundation science program in 1932 and direct the strategy of deployment of the large sums which that courageous and imaginative institution made available. It was indeed a large sum, for between 1932 and my retirement from the Rockefeller Foundation in 1959 the total of the grants made in the experimental biology program which I directed was roughly ninety million dollars. [Note: Somewhere between $900 million and $2 billion today depending on what he meant]
There is some purely factual basis to support the views of the preceding paragraphs. When I read Jim Watson’s exciting account of the discovery of the structure of DNA, and came, page after page, to the names of the individuals who had played leading roles, I was struck by the fact that all these names had been written down by me, time after time, in my Rockefeller Foundation diary, and indeed also in recommendations I had made to the Rockefeller Foundation Board of Trustees. As I read on in the Watson book, I jotted down the names of what seemed to be the most significant actors in the play. I wrote down thirteen names in a first and most important category, and fourteen others, who were somewhat less importantly involved. And of these two lists, every person in the former and more significant group had received assistance from the Rockefeller Foundation. All but three of the second group of fourteen had also received Rockefeller Foundation assistance.
Recently President George W. Beadle of the University of Chicago identified eighteen of the Nobel Laureates, over the period of 1954 to 1965, as having been involved in one or another aspect of molecular biology. The mere fact that fifteen of the eighteen had received assistance from the Rockefeller Foundation is not especially significant; for if they are such outstanding scientists it ought to be easy to identify them for aid. So it is much more noteworthy that the Rockefeller Foundation assisted every one of the fifteen before he received the Nobel Prize, and indeed on the average over nineteen years in advance.
Two powerful streams of thought have converged to form the present discipline of molecular biology — the flow of structure studies which recognize physical laws as basic and sufficient for the understanding of the form and function of parts of a living system, and the considerable flow of studies in the genetics of phage. The work in phage genetics was to a great extent developed under the leadership of Max Delbrück and his associates. Delbrück was originally trained as a physicist. Watson, one of the two architects of the structure of DNA, is a biologist originally trained in phage genetics.
To substantiate the claim of the Rockefeller Foundation’s influence upon the emergence and development of molecular biology, I have stated some facts about the record of grants to those scientists who led in this development. I can add to this some direct evidence from certain leading recipients of this aid.
Not long ago, I wrote a number of leading scientists who had been involved in the development of molecular biology, asking for their opinion as to the most satisfactory definition of the phrase “molecular biology,” and also raise some questions as to the ways in which this field came into being. In reply, Delbrück [likely the most influential early researcher in the field] wrote me: “I can only testify as far as I am concerned and here very strongly and unambiguously: without the encouragement of the Rockefeller Foundation received in 1937 and their continuing support through the mid-forties I believe I would hardly have been able to make my contributions to biology.”
As a part of this same exchange of correspondence about molecular biology, I received a letter from Sir W. Lawrence Bragg, the younger of the father-and-son team that received the Nobel Prize in 1915 for their determinations of crystal structure by X-ray diffraction techniques [and the head of the Cavendish Laboratory Watson and Crick would research in]. In this letter Sir Lawrence said, “concerning the part the Rockefeller Foundation played in helping the ‘Cambridge School.’ Your help came at a vital time just before the war when I was trying to find some way of supporting Perutz’s work [Max Perutz was the chairman of the Laboratory of Molecular Biology at Cambridge] and it was continued after it. This school was responsible for DNA, for the first protein structures, for the first understanding of virus structure, and for work on muscle. The extent to which the X-ray analysis of protein was pioneer work is shown by the fact that only now, twelve years after the trail was beaten at Cambridge and the Royal Institution, has any other research center succeeded in getting a protein ‘out.’ I am allowing myself to put this so strongly because I think that the Foundation’s help made an outstanding difference to these advances.” Perutz has also written me, “…the Cambridge work on the structure of large molecules would never have got off the ground but for the Foundation’s support.”
The Rockefeller Foundation has, I firmly believe, a solid and authoritative basis for taking satisfaction in the role it played in emphasizing, over a period of over a quarter of a century, the support of research in quantitative biology.
The Natural Sciences Division Before Weaver
To understand how things changed under Weaver, we first need to understand how the Rockefeller Natural Sciences Division operated prior to his arrival.
The Division used to be more…normal. “Normal” in that they more or less opened up their coffers to all comers, heard their pitches, and gave the money to whatever pitches they found most compelling or exciting. It was a generalist fund, for the most part, and, in the budgets, the money was earmarked for specific projects/labs/research areas — usually at universities. Even so, the funding was general enough by today’s standards that a Rockefeller grant was closer to what we’d know as funding a “person or department head, not a project.”
Rockefeller’s grants to individuals/departments tended to be for things like the exploration of some very general process with some specific-ish method. For example, the following is one of Caltech’s grants from the 1933 Rockefeller Foundation Annual Report:
The special need of the department at the present time is for additional personnel to undertake studies of the biological stages that are found between the genes in the fertilized egg and the finished characters of the organism. Between the initial stage, which is the province of cytology, and the final stage, which is the subject matter of genetics, lies a significant and unexplored region. The Foundation's grant will make possible investigations in this field.
This grant was made in Warren Weaver’s first year with proper control of the fund. And, while one can (maybe) make the argument that the scope of the grants was a little different under Weaver, it was largely the same in providing broad funding that trusted the scientists to deploy the money and change directions as they saw fit.
What did change? Well, Weaver came in with a thesis, a strong one. And, while many capital allocators have theses on future trends that make headlines all the time, few really put their money where their mouth is in the extreme fashion he did. Weaver was not just willing to increase funding in the area in which he had conviction in some moderate way. He was willing to divert funding from almost all other (very promising) areas of science to this one particular area of focus.
To some, this might make Weaver seem like a betting man. And if he was, he was a historically great gambler. But I’m not so sure Weaver would see it that way. He would not have characterized this allocation of capital as “going all in” on one area, but, rather, as the kind of responsible specialization that most people adopt in normal, private sector business. This was also the kind of specialization that Karl Compton, an intellectual leader of the research profession at the time, felt that research departments should put into practice to optimize results.
Over the next 20 years, exactly how Weaver went about this long-term commitment to funding, on the face of it, kind of weird science — with few ground-breaking public results in the field’s early years — is invaluable learning material. Not only did his strategy prove that an organization could reliably create scientific branches, but his work can also serve as a playbook for modern scientific grant funders looking to do the same.
Weaver’s Thesis: A wave not yet gathering its strength
In 1932, Weaver was very surprised when the Rockefeller Foundation invited him to come to New York City to interview for the job as the head of its Natural Sciences Division. The interview came about because of a recommendation from Max Mason, one of his undergraduate mentors who later became his collaborator. Mason became the Rockefeller Foundation President in 1929 after having run its Natural Sciences Division.
In the interview for the role — which Weaver felt very unqualified for but also believed was too interesting to pass up — Weaver spoke of how he was “convinced that the great wave of the future in science, a wave not yet gathering its strength, was to occur in the biological sciences. The startling visions that were just beginning to open up in genetics, in cellular physiology, in biochemistry, in developmental mechanics — these were due for tremendously significant advances.” Elaborating on his hypothesis at the time, he continues:
I strongly felt that the Rockefeller Foundation ought to undertake a large and long-range support of quantitative biology.
This was by no means a uniquely inspired conviction, for others had the same idea, notably the German physiologist and Nobel Laureate Otto Warburg, who had written: “…the most important problem in biology is to obtain an understanding in physiochemical terms of the processes — and the substances which take part in the processes — that occur in the normal living cell.”
The idea that the time was ripe for a great new change in biology was substantiated by the fact that the physical sciences had by then elaborated a whole battery of analytical and experimental procedures capable of probing into nature with a fineness and with a quantitative precision that would tremendously supplement the previous tools of biology — one can say “the previous tool” of biology, since the optical microscope had furnished so large a proportion of the detailed evidence.
Even at the time, more than 35 years ago [written around 1968], one could identify some of the procedures and the instruments that were ready to be applied more intensely to basic biological problems. Although a practical working instrument had not yet been built, it was known that a microscope using ultraviolet light could discriminate detail about ten times as fine as that analyzable by a microscope using ordinary light. Indeed the wave aspects of quantum theory indicated that an electron microscope — although working models were then some years off — could reveal details at least a thousand times finer. More indirect ways of analyzing structure — extensions of the ordinary processes of seeing — were soon to be available through the use of X-ray and electron diffraction studies.
In addition to new ways to see in greater and more revealing detail, there was a rich promise of new ways to separate out the constituents of complicated biological systems such as blood and the other fluids of the body. The supercentrifuge of the Swedish chemist Theodor Svedberg, for example, was already available.
When one today looks through the massive annual issue of Science which is devoted to equipment, he realizes the tremendous range and power of the instrumentation — much of it employing automatic electronic techniques — now available for quantitative experimentation in biology and medicine. This was of course not foreseeable in any detailed way during 1931-1932. But enough was discernible to convince one that biology was about to have the tools to enable it to enter upon a new era.
Although I was convinced that the Rockefeller Foundation ought to move in this direction, it seemed even clearer to me that I was not qualified to direct such a program. I told this emphatically to the top officers of the foundation. But I was enthusiastically convinced of the importance of moving in that direction, and because I did have the necessary background knowledge in physical sciences, they somewhat rashly, as it seemed to me, offered me the directorship of that division of the Rockefeller Foundation dealing with all aspects of sciences other than professionally medical.
While Weaver is being a bit modest about his foresight, his conviction is obviously strong and there is sound reasoning behind it. He was a very serviceable research physicist — although his degree was in mathematics — and had been paying close attention to related fields, as many of the curious researchers of the day did. He had seen how physics instrumentation and models had played a massive part in the development of the field of chemistry — to the point where the two fields became so intertwined that it was ambiguous whether certain Nobel Prizes should be awarded for physics or chemistry. He saw that the field of biology was similarly poised to build on the prior generation’s explosion in physics’ ideas and methods.
Weaver saw, or was pretty sure at least, that there was significant “complementarity” — Bohr’s word — between the two subjects that could be leveraged. However, this complementarity was much less obvious to see than it was for physics and chemistry. Even in the mid-1940s, over ten years after Weaver’s interview, many physicists who would make the transition to molecular biology were just beginning to consider the implications of their methods in the biological sciences after reading books like Schrödinger’s What is Life? — which did not really make any practical advancements but did excite many physicists into thinking about the field. Even in the mid-1940s, physicists felt like they were on the very early wave of a very new trend when dipping their toes into this field (more on that later). Nevertheless, in 1932, Weaver saw it. A few select individuals like Otto Warburg saw it. But this was not a common opinion.
Surprised he had even been offered the job, Weaver felt like he had nothing to lose in taking it. Weaver knew for a fact that he was not a “first-rate man” when it came to physics. In his own words:
I lacked that strange and wonderful creative spark that makes a good researcher.
How did he know that exactly? Well…because of Max Mason. Weaver and he were collaborators at the University of Wisconsin. And, while Weaver felt he was a solid contributing member of the duo, he believed that around 95% of the actual new ideas the duo generated sprung from Mason’s head. Weaver was helpful in that he could work longer hours to work things out and help keep Mason on a schedule — which Mason did not do on his own.
Weaver, however, did not have that “wonderful creative spark” he needed to be a great researcher, but he did have a spark of something. In New York, working at Rockefeller, it would truly flourish.
The Weaver Approach: True specialization in an extremely young field
A philanthropy’s annual budget can tell you far more than any vision statement what exactly they value. To get to the bottom of what differentiated Weaver’s approach, there is no better place to go than Rockefeller’s Annual Report — which includes its budget and detailed narration of the year’s decisions.
Rockefeller’s yearly reports are shockingly enjoyable to read. I read all of their annual reports — the Natural Sciences sections at least — from around 1929 to 1955 to really understand how the money ebbed and flowed under Weaver’s direction as well as before he got there. And, frankly, the budgets speak for themselves. The images of a single year’s budget, shown in the coming section, tell the story in a nutshell.
While, of course, there is a lot of nuance that you’d need to read at least five years worth of budgets to fully appreciate, the evidence from just one year’s budget should present a stark enough contrast to give you a pretty damn good idea of the “Weaver Approach.” On paper, the language of the thesis that Weaver shared with the Rockefeller Foundation doesn’t seem stronger than the “vision statements” you often hear philanthropic heads spout off. But what made him, and Rockefeller, so different was that he was willing to divert almost all his Division’s money away from other promising areas of the natural sciences budget to seed this young area with sufficient funding.
Rockefeller’s 1933 Natural Sciences Budget: New vs. Old Appropriations
Prior to Weaver’s arrival, the Rockefeller Foundation had given some money to molecular biology-related grants — like those to Linus Pauling and Caltech. But it would not be unfair to characterize their granting as “spread around” across many scientific areas.
This state of affairs should be unsurprising because 1) “spread around” is a typical state of affairs for scientific grant makers and 2) if Weaver was willing to bet his whole interview on supporting one new area of science, it is not surprising that the Foundation at least had a passing interest in the field if they were willing to hire the physicist with the oddly specific interview. But with Weaver there, the Natural Sciences Division began to give to “quantitative biology” at the expense of all other things. They had the conviction to deal with the tradeoffs.
Below is a snapshot of the Division’s “new appropriations” in 1933. New appropriations are the brand new donation areas that the Division chose to fund in that year. After, I’ll share a snapshot of the “former appropriations” from the 1933 Annual Report. Former appropriations are the appropriations that the Division was paying out because they were promised from prior years — before Weaver’s time.
Taking a look below, the new appropriations for 1933 are very focused. Around a quarter of the money went to research programs dedicated to quantitative biology (under “Programs of Specific Concentration”); around 3% was set aside for earth sciences research; 46% for broader goals such as institution building in China and supporting journals and certain pieces of European science; and the rest was spent helping boost national research council fellowships.
The modern equivalents of these dollar amounts are something like $4 million, $400,000, $7 million, and $4 million respectively. Of course, that was only one portion of Rockefeller’s science giving. The Rockefeller medical sciences total budget — which was a separate pot of money — was $23.4 million in that year’s new appropriations and $31 million in payments on appropriations from prior years.
An additional $28 million in Weaver’s budget was earmarked for funding promises made in prior years. Below, I show a snapshot of these prior funding commitments in that same year. You see shades of Weaver’s interests in there, with line items such as those for Caltech and Cold Spring Harbor Laboratory, but it is comparatively very scattered. One difference between this budget and the budget above is very obvious: they don’t even attempt to break it up into subsections. It’s just…one long list. It seems they knew there was a little bit of everything in there.
This “little of everything” approach was not uncommon then, just as it is not uncommon now. It’s very tempting to put some money into an account earmarked for donations and say, “The most interesting projects from the scientists that are most exciting to us in any area that seems cool can win this money. All should feel welcome to apply!”
But that didn’t make much sense to Weaver at all. He writes in the 1933 Annual Report on the change of strategy being implemented:
During the years immediately following 1929, when The Rockefeller Foundation's program in the natural sciences was first organized, there was recognized, in selecting projects for aid, some preferential emphasis upon certain fields of interest; but the primary emphasis was not upon field but rather upon the outstanding leadership of the chosen men or institutions. In recent years, however, interest in certain definite fields has played the dominant role in the selective process. The opportunities open to the Foundation in the field of the natural sciences are, in fact, not likely to be met by supporting undertakings merely because they are sound scientific projects, or even because they are outstandingly good scientific projects. A highly selective procedure is necessary if the available funds are not to lose significance through scattering. Within the fields of interest decided upon, selection naturally continues to be made of the leading men and institutions.
The Rockefeller Foundation may have had more money than God by contemporary philanthropic standards, but no organization was above specialization. The Natural Sciences Division would pass up on the most impressive people and projects applying for money if they were not applying to work in the “fields of interest decided upon.”
In practice, it seems Weaver felt that the effect of the Division’s money was being diluted by this “scattering” — or, differently framed, that concentrating the grants in one scientific area would be super-additive.
How did Weaver justify choosing this specific area of specialization to Rockefeller?
What many would call “using the money to its utmost effect by funding the best ideas,” Weaver saw as a missed opportunity to concentrate the money in a way that could generate exponential returns in scientific knowledge — creating new scientific branches. In this case, the branches he wanted to create were at the then-fuzzy intersection of physics and biology. He didn’t have a name for it because these branches didn’t exist yet; but he had a good reason to believe 1) why they should exist and 2) that it would be a big deal if they did.
In the 1933 Annual Report, already having explained that the Natural Sciences Division would begin to specialize, Weaver attempted to outline a general model to justify why he would be choosing the area of concentration he chose. In his autobiography, Weaver seems to indicate that, as often happens in situations like this, he may likely have first decided to focus on molecular biology and the formal model for the justification came afterward. That’s how these things often go. However, given that annual reports are annual reports, in the report the mental model comes first — it helps create the allure of deducing the course of action from first principles. Weaver’s mental model, typified by some fuzzy language characteristic of many annual reports, went as follows:
The choice of fields of interest is influenced by several considerations. The field must contribute in a basic and important way to the welfare of mankind. It must be sufficiently developed to merit support, but still so imperfectly developed as to need it. It should be a field in which the contributions of the Foundation can play a significant role in producing and stimulating development that otherwise would not take place within a reasonable time.
It is obvious that the welfare of mankind depends in a vital way upon man's understanding of himself and of his physical environment. The problems of physical and mental growth and development and of reproduction of kind are of central importance to all individuals. Not only the well-being of present society, but even to a greater extent the well-being of the society of the future, depends upon a deeper understanding of the nature of these problems. Science has made magnificent progress in the analysis and control of inanimate forces, but it has not made equal advances in the more delicate, difficult, and important problem of the analysis and control of animate forces. This indicates the desirability of increasing emphasis on what may be called the vital sciences, or sciences dealing with the processes of life. These include the biological sciences, psychology, and those special developments in mathematics, physics, and chemistry which are fundamental to biology and psychology.
He then continues with what is likely where he actually started his thought process: why branch-creation at the intersection of biology and physics was practically possible. Essentially, he believed that the tools and mental models developed for fundamental physics in the preceding decade, which had also been fruitfully used in the field of chemistry, could go a long way in answering certain fundamental questions in very micro-level biology, such as answering questions related to genetics and inheritance.
He then asks a bunch of motivating rhetorical questions about how far science can go — which, once again, are often fixtures of these yearly reports — and follows with:
The past fifty or one hundred years have seen a marvelous development of physics and chemistry, but hope for the future of mankind depends in a basic way on the development in the next fifty years of a new biology and a new psychology. In selecting projects for Foundation aid in the natural sciences the major emphasis at present is upon certain fields of modern analytical biology.
There was a general — not necessarily quantitatively worked out — sense that society had gotten some massive return (100X?) out of what it had invested into the fields of physics and chemistry in the preceding decades. And there seemed, to Weaver, to be an obvious chance that this opportunity in quantitative biology could have a similar payoff.
He goes on to explain why earth sciences will be getting some attention — as something of a side project — while then-extremely popular fields like atomic physics and astrophysics would be ignored. The excerpt — and the choice itself — gives a lot of insight on what Weaver felt about funding popular areas.
It has not been judged feasible for The Rockefeller Foundation to support an extensive program, in the varied disciplines which study all aspects of the physical stage on which the drama of life is played. The field of the earth sciences (covering, for example, meteorology, atmospheric electricity and magnetism, earth currents, geophysics, etc.) has, however, been chosen to form a modest complement to the principal program in vital processes. Certain aspects of research in this field, particularly meteorology, have practical applications of high importance. Work in this field has the whole world as its laboratory, and for certain programs, necessarily organized on an international scale, aid is naturally sought from such organizations as The Rockefeller Foundation.
Research in the earth sciences has received little attention, being somewhat pushed off the stage by the more spectacular researches in atomic physics and astrophysics. It has been popular to work on the atom and on the cosmos, but there has been, relatively speaking, very little study of the less spectacular but important problems that refer directly to the earth. The situation is in some respects like that which prevailed in astronomy before there was emphasis on the desirability of the study of the sun as the nearest star.
Funding earth sciences and this quantitative biology stuff over things like atomic physics and astrophysics ran contrary to the scientific community . In previous years, Rockefeller had given substantial amounts of money to things like telescopes and accelerators. Now, if Weaver did give to popular physics-related projects in an area like accelerators, it was specifically with the tool’s under-explored biological use cases in mind. And it’s not like the accelerator trend in physics was passing, or was even considered a fad at the time. Recall the following graph tracking accelerator speeds. The start of exponential growth in accelerator speeds tracked in the graph was just starting in 1933 – look at the X-axis.
Yet, in 1933, Weaver already felt that Rockefeller’s sights should be shifting to under-explored pastures. This move by Weaver is not dissimilar to doing something like halting grants to machine learning research in 2014. If you were doing it under the justification that, “That stuff is overrated and will never bear much fruit,” then you’d look silly. But if your thought process was, “Bigger, more risk-averse funders and most researchers are already seeing this as an exciting field to jump into. Why are my dollars needed there?”, then you might be onto something — if you had more nascent research areas you were passionate about.
Weaver did.
How much of Weaver’s budget was spent on molecular biology?
Weaver was set to double down — in a literal sense, much more than double — on molecular biology. Other things would get funding, but “quantitative experimental biology” was the star. Earth sciences and non-bio-related fellowship funding were also shrinking. Weaver writes:
During 1933 the Foundation's allocation for earth sciences was sharply reduced because of the even greater urgency of the biological program. On a diminished scale also, the Foundation continued to give such basic and general support to the natural sciences as is furnished by fellowships and by grants in aid of research.
In the coming years, molecular biology’s role in the Natural Sciences’ portfolio would continue to become even more concentrated as the Division steadily unburdened itself from prior years’ commitments. In that first year, only a quarter of the budget was allocated to molecular biology — a massive increase in size, but nothing close to what it would soon grow into. Over the next five years, as Weaver observed the progress of his early bets and more funds made themself available, he must have felt that there was a strong enough proof of concept to continue on with the strategy. By 1939, as we see in the Annual Report’s budget (below), he had increased funding in the area to account for around 79% of the Division’s yearly appropriations.
According to my calculation done using the 1939 budget and the Annual Reports narration of the line items, around 79% of the money was given to molecular bio that year. And that ballpark, 80% of appropriations going towards molecular biology, was no one-off occurrence. The total balance was at about that level for years. In the 1953 Annual Report, Weaver confirms this back-of-the-envelope math, reflecting:
For about twenty years modern experimental biology received main emphasis in the program of this division of The Rockefeller Foundation. In fact, about 80 percent of the financial support recommended over the period 1932-1952 was devoted to various aspects of modern biology.
Weaver was not all-in, but molecular biology was clearly Rockefeller’s scientific Priority A and B. The Natural Sciences Division was, in effect, a molecular biology fund plus side bets and pet projects.
The Early Culture of the Field
Even as late as 1947, fourteen years after Weaver began this grand funding experiment, the field of molecular biology felt young. After all, he did choose to fund the field because it was, in 1933, just an idea and wouldn’t have been desirable to other funders — in spite of its potential impacts. But just how nascent was this field at the time Weaver began to heavily invest in it?
Many researchers in related areas didn’t even begin hearing about molecular biology, let alone consider a transition to it, until over a decade after Rockefeller had begun its heavy commitment. Just how under-the-radar molecular biology went for the first (almost) two decades of its existence is well-captured by the autobiographical accounts of thirty top researchers in the book Phage and the Origins of Molecular Biology — published by Cold Spring Harbor Laboratory Press. Each chapter contains an account of one influential early molecular biology researcher, explaining how they came to the field or how some of their well-known experiments came to be. The unifying thread holding the book together is quite simple: Max Delbrück. In fact, the book was published in honor of Delbrück’s 60th birthday. The goal was to share the stories of researchers whose projects or research direction were influenced by Delbrück in some way — and he did a lot of influencing.
So, before I start sharing accounts from that book, I should briefly explain who Delbrück is.
Delbrück’s degree was originally in physics. As a theoretical physicist, his interest was first piqued in biology when he received a Rockefeller Fellowship to visit Niels Bohr in Copenhagen (a couple years before Weaver’s time at Rockefeller). It was Bohr who first interested Delbrück in approaching the field of biology with his “complementary” physics toolkit. For several years afterward, he would publish mostly physics-related work. But, in 1935, he co-authored a very interesting paper on the nature and structure of gene mutation. Weaver’s Division, 1) already having Delbrück on the payroll/being aware of him, 2) constantly being on the lookout for exciting opportunities in experimental biology, and 3) looking to help displaced European scientists, jumped at the opportunity to offer Delbrück a Fellowship. They found Delbrück a spot at Caltech — Caltech already had several projects underway in molecular biology which were largely Rockefeller-funded. This move to Caltech would have surely been considered a strange career move for the promising Delbrück: theoretical physicists were not a common feature of biology laboratories. Regardless, Delbrück went and made great use of his physicist’s toolkit, working in their experimental biology program. Pleased with his work, the Foundation extended his grant, and later, helped facilitate a full-time US position for Delbrück at Vanderbilt by fronting a portion of his salary there. He would publish a trio of papers in 1939-40 that were pivotal to the early growth of the field and, more importantly, be a fantastic teacher/mentor/recruiter to dozens of early scholars entering into the field. That’s why they were able to write an entire book solely containing prominent researchers’ accounts of Delbrück’s influence on them and their work — he was seemingly everywhere.
Now, back to the point: what the field of molecular biology was like up to the mid-to-late 1940s, inspired by accounts from Phage and the Origins of Molecular Biology.
The first account of how small the field was comes from Salvador Luria, a relative unknown at the time of his first Fellowship who, at the time, came loosely recommended by Enrico Fermi. He received his Rockefeller Fellowship to do research at Columbia in 1940. He would go on to be a close collaborator of Delbrück and later win a Nobel Prize.
Twenty-five years ago, when Delbrück and I first met, we were probably the only two people interested in phage from the point of view of “molecular biology.” Our correspondence between 1940 and 1943 dealt, more often than not, with the problem of attracting the interest of geneticists, biochemists, and cell physiologists to the dimly glimpsed green pastures of the promised land. The first phage meeting, in Nashville, Tennessee, March 1947, attracted eight people (M.H. Adams, T.F. Anderson, S.S. Cohen, Max Delbrück, A.H. Doermann, A.D. Hershey [also a Nobel winner], M. Zelle, and myself).
Even in 1947, 14 years after Weaver took over, the conference on phage in molecular biology was only eight people. As early as 1943, the total number of researchers in the area was two. Of course, there were far more than eight people studying molecular biology as a whole in 1947. But there were so many important open questions, and so few people in the field, that each particular area often had only a small cadre of individuals working on it.
Particularly in the earlier years of this branch-creating approach, it was the researchers particularly excited by taking a risk and carving out the true frontier of a field that found their way into the work — often powered by Rockefeller funds. Two researchers of this variety were George Beadle and Boris Ephrussi. The duo, at Caltech, saw that there was a problem: the organisms favorable for genetic study — such as fruit flies — were very different from the organisms that were favorable for embryology — such as sea urchins. Beadle wrote, “We thought something should be done about it and finally proposed that we each gamble up to a year of our lives trying to do it.” The two met in the first place because the Rockefeller Foundation had funded Ephrussi, whose lab was in Paris, for an extended visit to Caltech.
They decided to take this “gamble” — one year of their lives — at Ephrussi’s lab in Paris. The Rockefeller Foundation could not give a Fellowship to support Beadle’s trip on such short notice because they had never met him (meeting candidates was possibly the fellowship’s only strict requirement). But the Caltech department, heavily funded by Rockefeller, was able to cover Beadle’s salary while he was in Paris with Ephrussi. (Rockefeller would later fund Beadle to continue his work at Stanford.)
It was in this year that the two began to produce experiments with Drosophila (fruit fly) eye transplants that began to set the stage for the “one gene, one enzyme hypothesis” which was extremely important to the field at the time. When they arrived in Paris, they got right to work. And, as is so common when you read the scientific histories of these (very) early researchers carving out branches, it was scrappy and disorganized work. The individual’s personalities were all over the science. Beadle writes:
I arrived in Paris in May, 1935, and we immediately began to attempt to culture Drosophila tissues. This proved technically difficult; so, on Ephrussi’s suggestion, we shifted to transplantation of larval embryonic buds destined to become adult organs. We sought advice from Professor Ch. Perez of the Sorbonne, who was a widely recognized authority on metamorphosis in flies. He said we had selected one of the worst possible organisms and that his advice was to go back and forget it. But we were stubborn and before many weeks had devised a successful method of transplantation. The first transplant to develop was an eye. It was the occasion for much rejoicing and celebration at a nearby café…
…
Before long, we had established the existence of two diffusible eye-pigment precursors that we believed to be sequential in the formation of the brown component eye pigment…This was the beginning in our minds of the one-gene-one-enzyme concept, but we did not then use that expression.
That was the beginning of a thread of knowledge that would not begin to properly unravel for several years — but an important one, nonetheless. In these very early years of branch-making work, just getting a thread started is a massive accomplishment, because usually one cannot even be sure that there is one to be found beforehand.
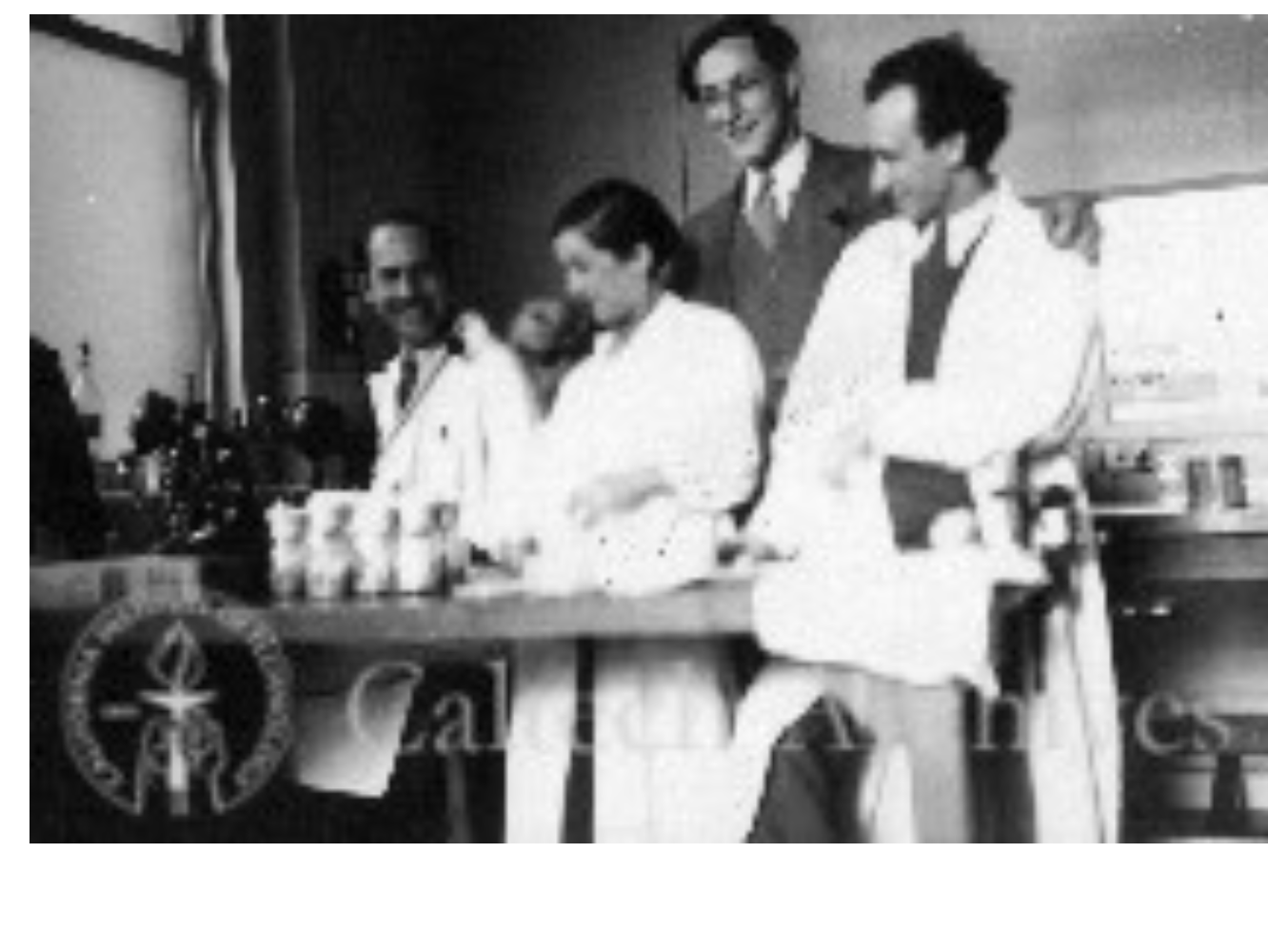
About five years later, Beadle would make fruitful use of the beginnings of this idea which had begun in Paris. He describes the moment it hit him. It happened as he was sitting in a fellow professor’s class at Stanford — in the very early years of branch-creation in a field, sitting in on the classes of professors from adjacent subjects can be extremely helpful. Beadle writes:
In 1940 we decided to switch from Drosophila to Neurospora. It came about in the following way: Tatum was giving a course in biochemical genetics, and I attended the lectures. In listening to one of these — or perhaps not listening as I should have been — it suddenly occurred to me that it ought to be possible to reverse the procedure we had been following and instead of attempting to work out the chemistry of known genetic differences we should be able to select mutants in which known chemical reactions were blocked. Neurospora was an obvious organism on which to try this approach, for its life cycle and genetics had been worked out by Dodge and by Lindergren, and it probably could be grown in a culture medium of known composition. the idea was to select mutants unable to synthesize known metabolites, such as vitamins and amino acids which could be supplied in the medium. In this way a mutant unable to make a given vitamin could be grown in the presence of that vitamin and classified on the basis of its differential growth response in media lacking or containing it.
There was never any slightest doubt that this approach would be successful — in my mind at least — for we had complete confidence in the one-gene-one-enzyme hypothesis.
Vital gains in knowledge had the potential to come almost instantaneously from any of the fields that were related to this fuzzy knowledge-space that was molecular biology. Leveraging related areas of research knowledge was vital to the field’s early growth — for all of its researchers. Rockefeller’s two biggest institution-based investments embodied this. The Rockefeller grants that supported molecular biology research at Caltech, in various departments, were implemented in a remarkably integrated fashion. And, every summer, Rockefeller funded free stays and use of the research facilities at Cold Spring Harbor Laboratory where researchers from a variety of related fields were brought into contact with molecular biology. Here, they were given a crash course in doing research in the subject and able to see what their fields’ toolkits could contribute to the growing movement.
Firstly, to say more on the Caltech grants. Emory Ellis, a former grad student at Caltech, writes of how integrated the research operation there was across all disciplines:
In the mid-1930’s Thomas Hunt Morgan was the chairman of the Biology Division of the California Institute of Technology, and the leader of its group of geneticists. In another part of the Institute, physicists were studying high-voltage X rays, and physicians associated with them were examining the effects of these radiations on human cancers. In the Chemistry Division, whose chairman was Linus Pauling, the tools for elucidation of molecular structure were being applied to proteins. In this small institution, these lines of research did not proceed in isolation from each other.
The importance of interdisciplinary communication is more generally appreciated today than thirty years ago, but it has always been a feature of research at Caltech. It played a part in the initial research at Caltech in bacteriophage, which came about through studies in the biochemistry of malignant tumors. Since some tumors were known to be transmissible by cell-free filtrates, it appeared that more knowledge regarding the nature of filtrable viruses would be helpful in understanding these, and perhaps other malignancies. Bacteriophage was the filtrable virus chosen for study as a model system.
Knowledge from other areas was heavily used to help shape one’s own research direction/research questions in the early years of the field — particularly at a place like Caltech. The experiments of the 1930s felt “simple and crude” to Ellis as he looked back on them almost 30 years later. But so many of them, while crude in retrospect, were only made possible in the first place through resourceful combinations of skills and knowledge from multiple fields.
And, at Cold Spring Harbor, things were also dynamic. Visconti — who would effectively abandon research after six hard years of working in the field — describes the kind of dynamic research environment Cold Spring Harbor was during the summers:
I hope to manage to portray faithfully the thrill we sensed at Cold Spring Harbor of working on the brink of new discoveries in a practically new science in the early 1950’s.
For somebody interested in testing simple ideas in science, working at the Carnegie Institution in Cold Spring Harbor between the years 1950 and 1953 was a unique opportunity. Life was easy-going and very informal, work schedules completely free, under the benevolent supervision and constant encouragement of M Demerec. One could obtain technical help of check on a literature reference in the library at any time of day or night. The field of phage genetics was developed enough that all sorts of experiments could be planned and discussed on the beach with people of different backgrounds, and then performed in the laboratory in the following days. It was enough to expound your ideas to Alfred Hershey or Evelyn Witkin to get an impression such as “It sounds good” or “It might be feasible,” and have all the materials prepared for the following day, along with some advice on the methods to follow. Collaboration was complete and the atmosphere very friendly.
And this was not some wonderful but out-of-reach place set aside for only elite members of some special group. This was the place where the early grinders of the field, such as Delbrück and Luria, brought young researchers, generally getting Ph.D.s in other subjects, to receive a crash course in the field and help teach them to contribute to it using their own toolkits. The people were very intensely interested in the work, but the structure and interactions, as described above, were lax and fun. The following is a photo of Delbrück, the field’s spiritual leader, as the lead in the lab’s production of A Midsummer Night’s Dream.
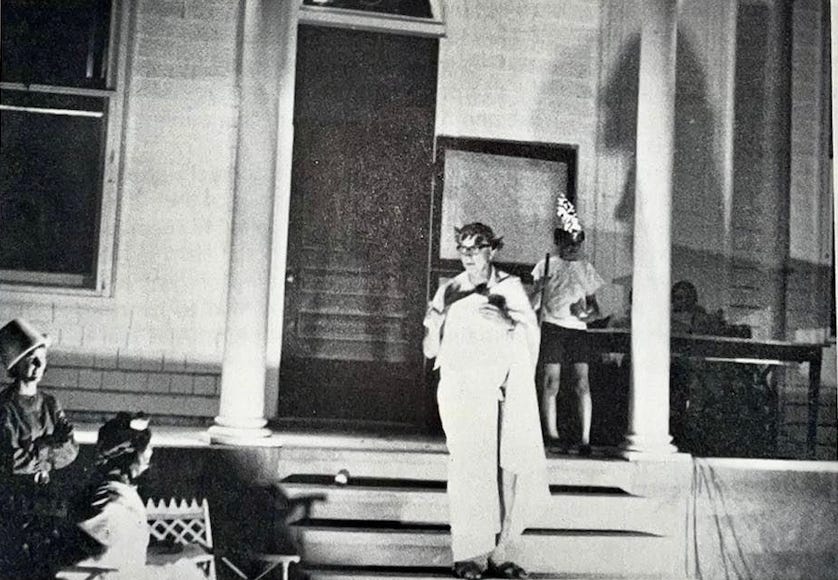
The people who gravitate to extremely early branch work are almost never very serious people. Curious? Extremely. Intense? In their own way, usually. But serious or formal? Almost never. The work just wouldn’t speak to them. The work has too much of an element of child’s play/fiddling/uncertainty.
Luckily, the same early researchers who could play Shakespearean characters brought some of that same energy to recruiting. A.D. Kaiser writes about how he found his way to Delbrück, Cold Spring Harbor, and biology in general:
Delbrück’s first entered my life in the form of the chapter heading “Delbrück’s Model” in Schrödinger’s book, “What is Life?” I read that book at an impressionable age, while still a graduate student in pre-transistor solid state physics at Purdue University. Not long afterward, at a meeting of the American Physical Society, at Bloomington, Indiana, a friend took me to visit the home of a former coed classmate of his. Her husband not only knew Delbrück personally, but even pulled a snapshot of him out of a drawer. I could not have been more impressed. The husband’s name was Salvador Luria, and it was not long before he had persuaded me to enroll in the phage course at Cold Spring Harbor. Thus I was suddenly plunged into the biology business.
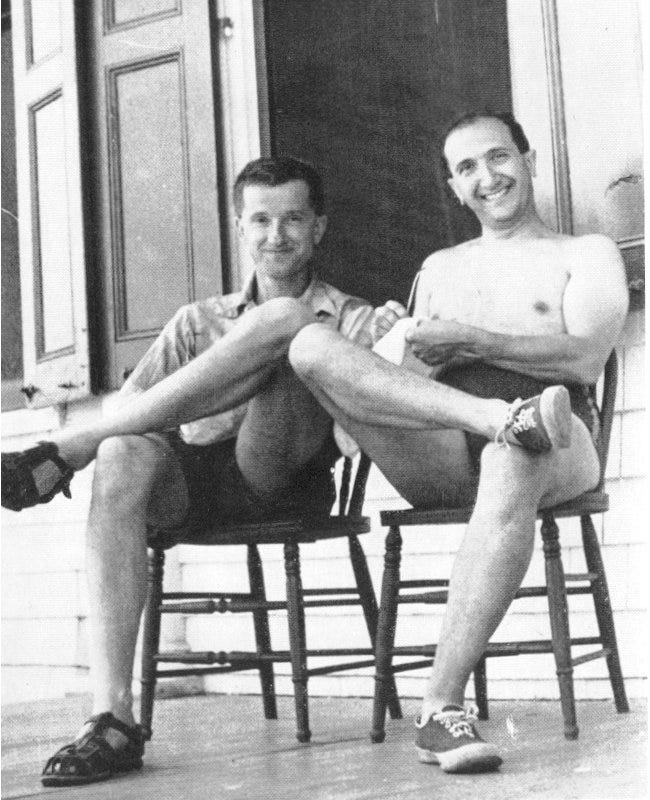
Reading through the accounts, the whole enterprise has the feeling of the “putting together your crew” portion of heist movies. Old friends approaching old friends with a weird idea that these Rockefeller guys might fund, in-the-know mentors notifying young researchers that there might be a chance to make their name in a speculative area, some guys who have been around for a little longer laying the groundwork, and even…a past his prime (scientific) elder statesmen watching a tennis game at the faculty club, pondering what (if anything) will come of the rest of his scientific days.
This was Leo Szilard’s pastime at his post-World War II academic home, the University of Chicago. The atomic bomb was, in a way, Szilard’s brainchild. He was now a famous and respected physicist. He could research whatever he wanted in the field of physics and it would almost surely get funding. But what he wanted was not to research physics anymore. He wanted to do great research again. And he felt that biology, not physics, was what gave him the best chance to make another discovery that would be hailed as truly great. Aaron Novick writes of a conversation he had with his collaborator and mentor:
One spring evening in 1947, as we were leaving a meeting of the Atomic Scientists of Chicago, Szilard approached me and asked whether I would care to join him in an adventure into biology. Despite his caution to think his proposition over carefully, I accepted immediately.
Apparently he had been considering a move to biology for some time, in part because he saw that the era of excitement in nuclear physics, where one man could contribute significantly, had ended and in part because he sensed that biology was on the threshold of an era much like that of physics prior to World War II.
But at the time it was unheard of for biology departments to appoint physicists, or even chemists, to their staffs. Fortunately, Robert Hutchins, Chancellor of the University of Chicago, appreciated Szilard’s greatness and had to courage to appoint him as Professor of Biology and Sociology, with specific departmental affiliations to be worked out later.
All in their own ways, a small group of young people and more experienced researchers were finding their way into this developing frontier — with the looming hand of Rockefeller support seemingly everywhere. But, it should not be forgotten, it took ten to fifteen years for the field to build to the point that we read about in most of the above accounts. As the 1950s approached, there was a bit of a feeling that a wave was building — the wave that Weaver knew, in 1933, should rightfully occur with the right support.
This was was the payoff of years of hard work and staunch commitments by Weaver’s division. For years before that, progress was not so obvious. Those early courses of experiments, funded by Rockefeller, did not look like the kind of science you see in more developed branches. It would have been very easy for Rockefeller to abandon ship in the first five years. In spite of this, the Foundation stood firm. They knew this was all a part of the process.
Doing Science in the Dark: A field in its infancy
Experimentation and “progress” can look a bit strange in the very early stages of branch-creation.
This is the biggest reason why funding this kind of work takes an especially strong conviction in your thesis. As much as any other type of scientific work, you don’t really know where the work will end up or what the specific questions to ask even are. Not only does the scientist need to be inspired by the extreme ambiguity of the situation, but it also takes a special kind of funder.
The Rockefeller Foundation was an ideal match for the situation because 1) they trusted the researchers they funded to passionately pursue their work without much oversight and 2) they had a strong thesis that a field at the border of physics and biology should exist. This second point is extremely important when it comes to not doubting the process every step of the way. Almost none of the projects seemed to finish in the same vein that they started in. That was par for the course, as I’m sure Weaver expected. That is why the funding couldn’t have been for a specific course of experiments, the way it often is today, even if Weaver wanted it to be. Anything more specific would have been pointless.
The messiness of the whole process is everywhere in Phage. Thomas Anderson, who along with Luria was the first to identify a set of phage particles, opens his chapter of Phage writing:
As everybody knows, most discoveries in science are necessarily quite unexpected: during a planned study that is more or less routine one encounters a surprising result that must be explained. I would like to describe two or three such observations that I stumbled upon at about that time and which eventually helped us to understand some of the mysteries that surrounded phage structure and growth.
The subsection headings in the section of his “Identification of Phage Particles” chapter, which cover only one short time period of his career in the field, contain headings like: “Enter Luria”, “What Are They — Really?”, “Enzymes? No!!”, and “Sperm? Maybe?” It was all touch and go, even through the early 1940s — ten years after Weaver began this experiment. But that wasn’t things not going according to plan. That was just… science.
André Lwoff stumbled upon his Nobel Prize-winning discovery, outlined in Phage, through a series of determined failures and an accompanying “eh, what else can go wrong if we try something a bit random?” moment. He writes:
Our aim was to persuade the totality of the bacteria population to produce bacteriophage. All our attempts — a large number of attempts it was — were without result. Louis Siminovitch and Niels Kjeldgaard became very depressed, and even repressed…Yet I had decided that extrinsic factors must induce the formation of bacteriophage. Moreover, the hypothesis had been published already, and when one publishes a hypothesis, one is sentenced to hard labor. Finally, I am stubborn, so the experiments went on, day after day, and they continued to be desperately negative.
…
Our experiments consisted of inoculating exponentially growing bacteria into a given medium and following bacterial growth by measuring optical density. Samples were taken every fifteen minutes, and the technicians reported the results. They were so involved that they had identified themselves with the bacteria, or with the growth curves, and they used to say for example: “I am exponential,” or “I am slightly flattened.” Technicians and bacteria were consubstantial.
So negative experiments piled up, until after months and months of despair, it was decided to irradiate the bacteria with ultra-violet light. This was not rational at all, for ultra-violet radiations kill bacteria and bacteriophages, and on a strictly logical basis the idea still looks illogical in retrospect. Anyhow, a suspension of lysogenic bacilli was put under the UV lamp for a few seconds.
…
It was a very hot summer day and the thermometer was unusually high. After irradiation, I collapsed in an armchair, in sweat, despair, and hope. Fifteen minutes later, Evelyne Ritz, my technician, entered the room and said: “Sir, I am growing normally.”
She returned back every fifteen minutes with updates like, “I am still growing normally,” until, after about an hour, “Sir, I am entirely lysed.” This work, helping prove how induction works, would go a long way in explaining aspects of bacterial reproduction and enzyme production. Lwoff referred to this as the greatest thrill of his scientific career; while he had made important findings in other areas, he took a special joy in this work because “with induction we had been in complete darkness.”
While randomly throwing your hands up and irradiating something under the logic of, “well…we know it shouldn’t work…but I guess we don’t really know anything do we? If we did, we wouldn’t be in this situation. Why not. Let’s do it!” was not exactly the norm. But fumbling around in controlled chaos was. Controlled in that…I guess they were writing everything down. And they did know other science…just not this science, not yet anyway. And they did have theories/mental models for doing what they were doing. But it was very standard, with these small groups of researchers trying to get their bearings in young sub-areas, to change multiple parameters massively between one experiment and the next. After all, why not?
Changing one or two parameters (marginally) and leaving everything else constant from a similar experiment that worked in the existing literature seems to be the careful luxury of researchers that are already somewhere worth being — in terms of how much they know about a field. That kind of thing is what science looks like further along on a branch. In the case of a molecular biologist circa 1940, little improvements on knowing jack shit still left one with jack shit.
The researchers’ search process, in undertaking a course of experiments, had to be more ambitious. In practice, it was conceptually closer to a midpoint search algorithm: casting themselves out into open space and, of course, finding nothing in the beginning, but gradually building on the things they did learn from one experiment to the next. You find nothing each time, for a while, but find a kind of nothing that’s iteratively closer to something — even if it’s still nothing. Eventually, when it worked out, they’d strike upon something quite new and useful. Other times they’d find not much, and that was ok too.
Oftentimes, the learning was as much in the realm of learning by doing than stereotypical scientific learning. These practical learnings were often things related to how and why experiments, materials, and solutions behaved the way they did in this new area of science; the actual results of many of these finicky experiments were mostly useless. This element of learning by doing was a necessary stage of the process that would eventually allow the scientists to be able to run experiments that had stronger, more traditional scientific validity. After explaining how the publishing of the above results worked, Lwoff goes on to explain a separate course of experiments that talk a little about this learning by doing:
Irradiation of lysogenic bacteria with UV light can induce the vegetative phase of the bacteriophage. May I remind you that the first indication of induction came from the observation of a single bacteria in microdrops, that we held the medium responsible, and that we tried in vain to identify the responsible factor. Induction with ultra-violet light triggered a new series of experiments. It was finally found that thioglycolic acid and a number of other reducing substances are powerful inducers (Lwoff and Siminovitch, 1951a and 1952). Our experiments had been performed in a medium containing yeast extract. And everything went on beautifully until the sample of yeast was exhausted. The media prepared with another batch, and all other media, were devoid of inducing activity after addition of thioglycolic acid. A number of yeast extracts were prepared and one of them turned out to be “active” and others not. After numerous experiments it was found that the active medium lost its activity by treatment with 8-hydroxy-quinolein, and the responsible factor was identified as copper (Lwoff, 1952). Inactive media could be activated by the addition of copper. It is known that the oxidation of sulfhydryl compounds by copper yields hydrogen peroxide. As a matter of fact, addition of hydrogen peroxide to an organic medium induces phage development. Organic peroxides are inducers, as are a number of mutagenic/carcinogenic agents (Lwoff and Jacob, 1952). Thus an explanation of the apparently spontaneous production of bacteriophage could be provided. Bacteria produce reducing substances. If the medium contains copper, the reducing substances are oxidized and hydrogen peroxide is formed. Hydrogen peroxide reacts with organic substances. Organic peroxides are formed, which produce the alteration of the bacterial metabolism responsible for induction. Of course, this alteration could as well be the result of rare mutations of critical bacterial genes.
[Lwoff then goes on to explain the step-by-step mechanisms that they now understand, clearly, as a sub-field.]
Things are clear now, but have not always been.
In reading Phage, one is struck by the shocking percentage of useful insights that came not from the formal experimental setups, but in all the sub-explorations needed to learn things like understanding which yeasts did and did not do what when diluted, how to control temperatures in different settings, the right concentrations of fluids and substrates, etc. Painstaking explorations were required to end up with the right bacterium, right prophage, right medium, and correct conditions in the microdrops to learn what they actually wanted to learn.
Many of the groups that found success tended to be laboratory “generalists” to enough of a degree that they could innovate around their problems — sometimes even building equipment to specially fit their needs or because they could not afford to buy it. Sometimes, these generalists solved their way around an issue so well that they became known as “specialists” in the materials and methods they deployed to work their way through the original issue.
Creativity or a relentless can-do attitude (which I know sounds corny) can take one a long way when a field is at an early stage. The ability to constantly keep up with the literature counts for much less. This kind of opportunity is exactly what Szilard craved: ambiguities and open questions that could be worked through by lone researchers in many cases. It was similar to what physics was in his youth.
In this stage, the list of things you know is so small and the list of things you don’t feels terrifyingly large. The experimental and theoretical successes that advance the field may come suddenly, when they do come, but the hard and confusing process of figuring out what the right questions are and how to make experiments work is vexing you constantly.
It is unbelievably hard, on a human level, to find something that you don’t know is there to be found. To relate this to something readers might understand, when doing chess puzzles, endgames in particular, performance is far higher when individuals are told by the puzzle creators that there is a checkmate to be found — rather than simply saying “find the best move” without indicating a mate is on the board.
To do early branch-creating science, researchers and funders have to constantly exist and work well in this ambiguity.
Moving on: Why Watson and Crick’s discovery spelled a new chapter for Weaver’s division
When Warren Weaver chose to shift his division’s primary focus from molecular biology is just as interesting as how he helped build up the field in the first place. He chose to exit the field just as many felt it was entering its “classical era.”
Many view the research events described above, from around 1933 to around 1952, as something of a precursor to the massive scientific wins that were:
The Hershey-Chase experiments in 1952 that confirmed that genes were made of DNA.
Watson and Crick’s now-legendary 1953 work positing the double-helix structure of DNA.
To many, that was the start of the field just hitting its prime, the classical era of molecular biology. These were the type of discoveries that the entire field had been building toward, the type of knowledge that could be leveraged for all sorts of applications, and the reason that Warren Weaver had gotten into the molecular biology game in the first place. And, after this discovery, many of the field’s holes were rapidly filled, leveraging this vital knowledge. So, if you’re counting usable outputs or the total number of researchers in a field, Watson and Crick’s discovery was just the beginning.
But, from another point-of-view, Watson and Crick’s discovery marked the end of the great era of molecular biology. John Cairns, the lead editor of Phage which I have quoted so heavily from, wrote an interesting 1999 article in Nature titled “Last Days in Arcadia” that speaks to this point. It begins:
The entire genetic code had been worked out by 1966. In a sense, this was not really a discovery. Obviously there had to be some kind of code and, in the end, it was deciphered more by brute force than subtle argument, using short molecules of RNA to drive protein synthesis in a test-tube. But the table showing the meaning of each of the 64 possible triplets was the culmination of all that had gone before.
In the 13 years since Watson and Crick had revealed their model for the structure of DNA, the essential ingredients that handle biological information had been identified — DNA sequence, operons, repressors, messenger RNA, transfer RNA and ribosomes, plus the various classes of enzymes needed to make those ingredients. These were formidable discoveries, and they changed the science of molecular biology from an esoteric field inhabited by a small coterie into the discipline that has dominated biology ever since.
In those days, there were far fewer scientists. Conferences did not tread hard upon each others' heels, and a single symposium at the Cold Spring Harbor Laboratory in New York state could just about cover all of molecular biology.
Watson and Crick’s discovery changed the kind of science that could get results in molecular biology. They helped usher in an era where, given a sufficient amount of the biological systems in question were finally understood to a moderate level, “brute force” scientific exploration could yield dividends. By “brute force,” I think Cairns is referring to the kind of work that is now so common in the NIH-funding universe: pursuing projects that are mostly copies of things that have worked before with one or two parameters changed to some moderate degree.
With the field going from one of potential promise — for those with faith — to one that 100% was ripe for a generation of fast results, people flooded into the field. But, with this ushering in of a new era, it also became the kind of field that someone like Szilard was probably less excited by. Fields on older branches that are in the midst of their era of brute force/big science are not nearly as exciting to individuals looking to make their mark in history.
But, such is the game. This is what winning looks like. Weaver and the Rockefeller Foundation had been right. An unbelievable amount of the field’s success was underpinned by Rockefeller Natural Science’s money in some way. So, after their years of dedicated work — and probably looking crazy to the rest of the world — their bet was very publicly paying off.
What did Weaver’s Division do then?
Well…they began the process of moving on. To the world, this young and exciting field had just started. But, to Weaver, Rockefeller’s work there was mostly done. He writes in the 1953 Annual Report:
For about twenty years modern experimental biology received main emphasis in the program of this division of The Rockefeller Foundation. In fact, about 80 per cent of the financial support recommended over the period 1932-1952 was devoted to various aspects of modern biology. The remaining 20 per cent was divided about equally between support of science as a whole, and support of special projects outside the biological program, which were aided because of their unusual nature, outstanding quality, and special importance.
Over recent years, however, the Foundation has been developing an increasing interest in agriculture. Begun originally as an isolated experiment, involving activities in Mexico only, this agricultural interest has expanded in several respects.
…
The expansion of the Foundation's interests in agriculture and in longer-range food problems has entailed some curtailment of other divisional activities. Support for projects in experimental biology within the United States is being substantially reduced, although the Foundation continues to have an active interest in advancing research in such fields as genetics, plant biochemistry, and plant physiology, which will be of ultimate value to agriculture.
Molecular biology no longer needed a committed ally like Rockefeller with a vision of what the field could be one day. The field had arrived, and all of the more risk-averse, follow-on funders surely saw it too. Instead, moving forward, their money would go towards what was previously one of the Division’s side bets: agriculture. It is very possible that the 20% of the budget Weaver had been allocating to projects of “unusual nature, outstanding quality, and special importance” were, to some extent, scouting missions for what the Natural Sciences Division's next thing could be when they decided to move on from molecular biology. As we discussed earlier, one of these side bets in 1933 was Earth Sciences. Another, in 1939, was Vannevar Bush’s differential analyzer project — one of only three small side bets the division made that year. Maybe Weaver was considering the field of computing as a candidate for Rockefeller’s next big project at one point? Regardless, in the end, the Division pursued agriculture.
This agricultural work would explode in the coming years, eventually outgrowing the Natural Sciences Division with a budget and staff all its own that both exceeded those of the Natural Sciences Division. The eventual scientific claim to fame of the Agricultural Division: miracle rice, which is credited with saving millions of lives since its creation and widespread use throughout the world. The Division did it again.
Surely, Weaver could have deservedly basked in the glory of what his division helped create — continuing to fund molecular biology for another 15+ years while its applications were deployed into the world. But, just as Rockefeller’s 1933 budget showed us exactly what Weaver valued, the 1953 Annual Report does just as clearly: Weaver was not committed to molecular biology at the expense of all other things. He was committed to creating high-value branches at the expense of all other things.
The Division would not abandon its young branch — molecular biology — because it was not publicly producing in a way that would make headlines, but they would abandon their baby if they felt it didn’t need them anymore. There had to be some new, undiscovered branch out there in need of their support. The follow-on funders could take it from there.
For some people, like Weaver and Szilard, life’s too short to spend basking in the glory of what they’ve built. They’d rather do the damn thing again.
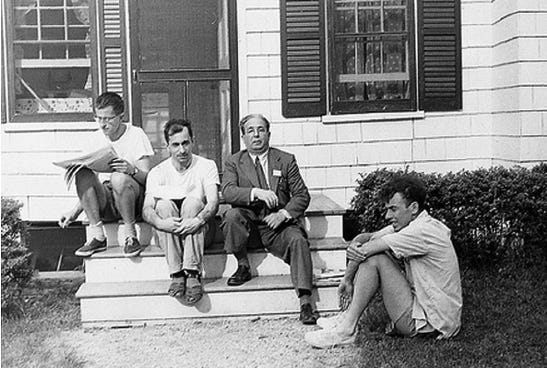
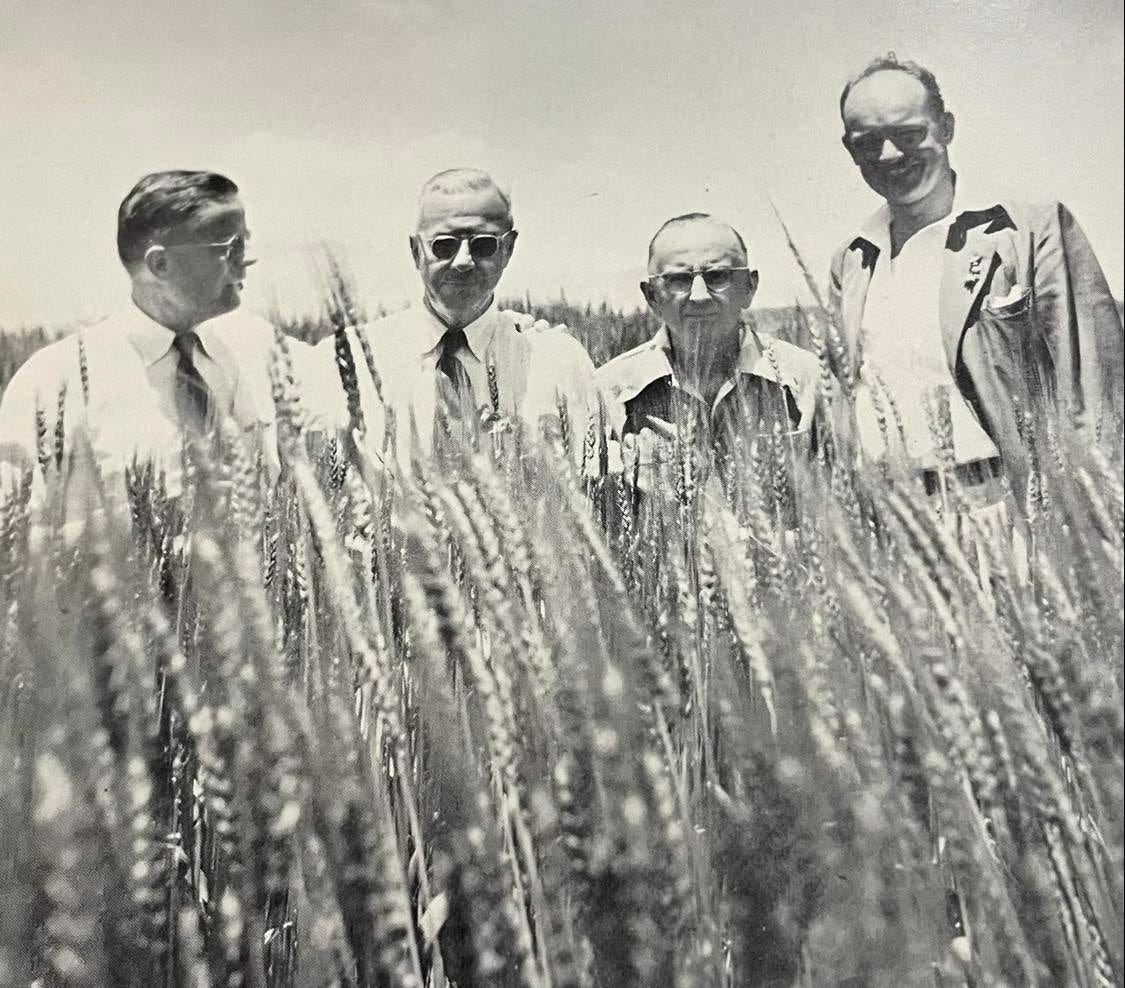
This is the end of the main piece. Stuart Buck and myself are writing a separate ‘How-To’ for individual funders and organizations looking to attempt branch-creation on their own. This will also contain additional historical details less relevant to the general audience, but very relevant to grant funders. We’re also hoping to have some bright researchers share some possible future branches in the piece that they think are worth looking into/funding! If you know any researchers who love weird and out there ideas, send them my way!
Subscribe if you don’t want to miss that. Also, feel free to reach out in the meantime. I’d love to talk!
Citation:
Gilliam, Eric. “A Report on Scientific Branch-Creation: How the Rockefeller Foundation helped bootstrap the field of molecular biology,” FreakTakes Substack. 2023. https://freaktakes.substack.com/p/a-report-on-scientific-branch-creation
Footnote
Books:
Phage and the Origins of Molecular Biology
Scene of Change: A Lifetime in American Science
1939 Budget Allocation Calculation
Doing the math on the 1939 budget, the following is the rough breakdown of the percentage of new appropriations to molecular biology in 1939 and how I did the approximate calculation. (This is the one I’d recommend you skip):
All experimental bio grants == $768,375
32,500 + 9,000 + 70,000 + 30,000 + 200,000 + 10,000 + 11,465.45 + 3,534.55 + 50,000 + 24,000 + 31,500 + 100,000 + 115,000 + 21,375 + 60,000 = 768,375
Fellowship grants to experimental bio = $100,000 (roughly)
In Fellowships, almost all of the RF’s $50,000 in allocated fellowships were molecular biology related. The NRC-funded ones — amounting to $180k — were more spread out. The fellowships were granted for research in the following subjects: zoology and botany, ten each; physics and astronomy, nine; chemistry, nine; geology and geography, seven; anthropology and psychology, six; and mathematics, three. We’ll conservatively say something like a quarter of those are molecular biology related for the sake of this exercise. So something like $100k of the total $230k in fellowships were molecular biology-related.
Non-biology-related general projects == $0
The three non-bio general projects add to
12,000 + 49,500 + 61,956.54 = 123,456.54
Zero of that was molecular bio related
Grants in aid to molecular bio = $144,000 (roughly)
For the grants in aid projects, all but three were molecular bio-related.
$160k in total. There were 58 in total. The three non-bio grants did look a little more expensive than average. We’ll say they were 10% of the money even though they made up only 5% of the projects. So roughly $144,000 of $160k was molecular bio-related.
In Summary: Roughly 79% of total 1939 grant dollars were given to molecular biology.
$1,012,375 in molecular biology grants out of $1,281,831.54 in total grants
Total natural sciences money allocated that year on purpose:
768,375 + 230,000 + 123,456.54 + 160,000 = $1,281,831.54
Total molecular bio money allocated that year:
768,375 + 100,000 + 0 + 144,000 = $1,012,375
On Gerald Holton's line continuation vs branching model. It reminds me of something I just came across yesterday, it was a piece by Nature on how if a scientist further moves away from the topic of his previous work, the fewer citations he will get.
If Holton's model can be downsized to an individual. then we seem to be promoting line continuations rather than branching even at the micro level.
On the topic of next big thing. For biology, it's definitely Bioelectricity. I mean it's at a more advanced stage than what Weaver saw with molecular biology in 1933. It's most prominent scientist (Dr.Michael Levin) is a well-established/semi-established figure by now. Though the field as a whole as whole is largely unexplored. (If the promises of its implications turn out to be true. Which I do believe in)
One a whole, largely speculative, but biology might benefit from a change of lenses i.e. replacing to some degree the reductionist- bottoms up framework that birthed up molecular biology and recentering the physiological-kind of top down framework.
As Denis Noble says, reductionist biology while giving us a lot of progress and wonderful discoveries in biology might have also turned us blind to lots of implications in biology that would be better viewed with different type of lenses.
I am very curious to know what a "loose recommendation from Enrico Fermi" was like 😀